Medical expert of the article
New publications
Mesenchymal stem cells
Last reviewed: 06.07.2025
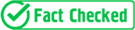
All iLive content is medically reviewed or fact checked to ensure as much factual accuracy as possible.
We have strict sourcing guidelines and only link to reputable media sites, academic research institutions and, whenever possible, medically peer reviewed studies. Note that the numbers in parentheses ([1], [2], etc.) are clickable links to these studies.
If you feel that any of our content is inaccurate, out-of-date, or otherwise questionable, please select it and press Ctrl + Enter.
Among regional stem cells, a special place is occupied by mesenchymal stem cells (MSCs), the derivatives of which constitute the stromal matrix of all organs and tissues of the human body. Priority in MSC research belongs to representatives of Russian biological science.
In the middle of the last century, a homogeneous culture of multipotent stromal stem cells of bone marrow was isolated for the first time in the laboratory of A. Friedenstein. Mesenchymal stem cells attached to the substrate maintained high proliferation intensity for a long time, and in cultures with a low seeding density after fixation on the substrate they formed clones of fibroblast-like cells that did not have phagocytic activity. The cessation of MSC proliferation ended with their spontaneous differentiation in vitro into cells of bone, fat, cartilage, muscle or connective tissue. Further studies made it possible to establish the osteogenic potential of fibroblast-like cells of the bone marrow stroma of various mammalian species, as well as their colony-forming activity. In vivo experiments have shown that both hetero- and orthotopic transplantation of colony-forming fibroblast-like cells results in the formation of bone, cartilage, fibrous and adipose tissue. Since bone marrow stromal stem cells are characterized by a high capacity for self-renewal and multifaceted differentiation within a single cell line, they are called multipotent mesenchymal progenitor cells.
It should be noted that over 45 years of fundamental research into mesenchymal stem cells, real conditions have been created for the use of their derivatives in clinical practice.
Today there is no doubt that all tissues of the human body are formed from stem cells of various cell lines as a result of the processes of proliferation, migration, differentiation and maturation. However, until recently it was believed that stem cells in an adult organism are tissue-specific, i.e., capable of producing lines of specialized cells only of those tissues in which they are located. This conceptual position was refuted by the facts of transformation of hematopoietic stem cells not only into cellular elements of peripheral blood, but also into oval cells of the liver. In addition, neural stem cells turned out to be capable of giving rise to both neurons and glial elements, as well as early committed lines of hematopoietic progenitor cells. In turn, mesenchymal stem cells, which usually produce cellular elements of bone, cartilage and adipose tissue, are capable of transforming into neural stem cells. It is assumed that in the process of growth, physiological and reparative tissue regeneration, uncommitted progenitor cells are generated from tissue-non-specific stem reserves. For example, muscle tissue reparation can be realized due to mesenchymal stem cells migrating from bone marrow to skeletal muscles.
Although such cross-interchangeability of stem cells is not recognized by all researchers, the possibility of clinical use of mesenchymal stem cells as a source for cell transplantation and a cellular vector of genetic information is no longer disputed by anyone, as is the multipotency of bone marrow stromal stem cells, which can be relatively easily isolated and expanded in vitro culture. At the same time, reports on the potential pluripotency of bone marrow stromal stem cells continue to appear in the scientific literature. As evidence, research protocols are cited in which, under the influence of specific transdifferentiation inducers, MSCs are transformed into nerve cells, cardiomyocytes and hepatocytes. However, some scientists have serious doubts about the possibility of repeated activation and expression of genes from the period of early embryogenesis. At the same time, everyone understands that if conditions are found for expanding the multipotency of mesenchymal stem cells to the pluripotency of ESCs, many ethical, moral, religious and legal problems in regenerative plastic medicine will be automatically resolved. In addition, since in this case the source of regenerative stem potential becomes the patient's autologous stromal cells, the problem of immune rejection of the cell transplant is also solved. The near future will show how realistic these prospects are.
Use of mesenchymal stem cells in medicine
In the clinic, the use of mesenchymal stem cell derivatives is primarily associated with the restoration of tissue defects that occur with extensive and deep thermal skin lesions. At the preclinical stage, an experimental assessment of the feasibility of using allogeneic fibroblast-like mesenchymal stem cells to treat deep burns was carried out. It was shown that bone marrow fibroblast-like mesenchymal stem cells form a monolayer in culture, which makes it possible to transplant them to optimize the regeneration processes of deep burn wounds. The authors note that embryonic fibroblasts have a similar property, but their clinical use is limited by existing ethical and legal problems. A deep thermal burn with damage to all skin layers was modeled on Wistar rats. The burn area was 18-20% of the total skin surface. The first experimental group included rats with a deep thermal burn and transplantation of allogeneic fibroblast-like mesenchymal stem cells. The second group consisted of animals with a deep thermal burn and transplantation of allogeneic embryonic fibroblasts. The third group was represented by control rats with a deep thermal burn that did not undergo cell therapy. A suspension of fibroblast-like mesenchymal stem cells and embryonic fibroblasts was applied to the surface of the burn wound using a pipette in an amount of 2 x 10 4cells on the 2nd day after burn modeling and excision of the resulting necrotic scab. After cell transplantation, the burn surface was covered with a gauze napkin moistened with isotonic sodium chloride solution with gentamicin. Bone marrow cells were collected to obtain MSCs with their subsequent induction into a line of fibroblast-like mesenchymal stem cells from adult Wistar rats from femurs. Embryonic fibroblasts were obtained from the lungs of 14-17-day-old embryos. Embryonic fibroblasts and bone marrow cells for obtaining MSCs were preliminarily cultured in Petri dishes at a temperature of 37°C in a CO2 incubator, in an atmosphere with 5% CO2 at 95% humidity. Embryonic fibroblasts were cultured for 4-6 days, while the formation of a monolayer of MSCs required from 14 to 17 days. Subsequently, MSCs were cryopreserved as the source material for fibroblast-like mesenchymal stem cells, which were obtained by defrosting and culturing MSCs for 4 days. The number of fibroblast-like mesenchymal stem cells formed was more than 3 times higher than the number of embryonic fibroblasts formed during the same cultivation period. To identify the transplanted cells in burn wounds at the cultivation stage, their genome was labeled using a viral shuttle vector based on recombinant adenovirus type V carrying the 1ac-2 gene encoding E. coli ß-galactosidase. Live cells at different times after transplantation were detected immunohistochemically in cryosections with the addition of X-Gal substrate, which gives a characteristic blue-green stain. As a result of dynamic visual, planimetric and histological assessment of the burn wound condition, it was established that already on the 3rd day after cell transplantation, significant differences in the course of the wound process appear in the selected groups. This difference became especially distinct on the 7th day after cell transplantation. In the animals of the first group, to which fibroblast-like mesenchymal stem cells were transplanted, the wound acquired a uniformly intense pink color, granulation tissue grew over its entire area to the level of the epidermis, and the burn surface significantly decreased in size. The collagen film formed on the wound surface became somewhat thinner, but it continued to cover the entire burn area. In the animals of the second group, to which embryonic fibroblasts were transplanted, the granulation tissue rose to the level of the epidermis of the wound edges, but only in places, while plasmorrhea from the wound was more intense than in the 1st group, and the initially formed collagen film practically disappeared. In animals that did not receive cell therapy, on the 7th day the burn wound was pale, pitted, necrotic tissue covered with fibrin. Plasmorea was noted over the entire burn surface.Histologically, the animals of the 1st and 2nd groups showed a decrease in cellular infiltration and development of the vascular network, and these signs of the incipient regenerative process were more pronounced in the rats of the 1st group. In the control group, signs of cellular infiltration of the wound were observed, the histological pattern of newly formed vessels was absent. On the 15th-30th day of observation, the area of the burn surface in the animals of the 1st group was significantly smaller than in the rats of the other groups, and the granulating surface was more developed. In the animals of the 2nd group, the area of the burn surface also decreased compared to the size of burn wounds in the rats of the control group, which occurred due to marginal epithelialization. In the control group, the burn surface remained pale in places with rare granulations, vascular asterisks appeared on it, there were islets of fibrinous plaque, moderate plasmorrhea continued over the entire burn surface, and a scab that was difficult to separate remained in some places. In general, in animals of the 3rd group, the size of the wound also decreased, but the edges of the wound remained undermined.
Thus, during a comparative study of the rate of wound healing using fibroblast-like mesenchymal stem cells and embryonic fibroblasts, as well as without the use of cell therapy, an acceleration of the rate of healing of the burn surface was noted as a result of transplantation of fibroblast-like mesenchymal stem cells and embryonic fibroblasts. However, in the case of using allogeneic fibroblast-like mesenchymal stem cells, the rate of wound healing was higher than with transplantation of embryonic fibroblasts. This was expressed in the acceleration of the change of phases of the regenerative process - the terms of cellular infiltration were reduced, the rate of vascular network growth increased, as well as the formation of granulation tissue.
The results of dynamic planimetry indicate that the rate of spontaneous healing of the burn wound (without the use of cell therapy) was the lowest. On the 15th and 30th days after transplantation of allogeneic fibroblast-like mesenchymal stem cells, the rate of wound healing was higher than with transplantation of embryonic fibroblasts. Histochemical method for detecting beta-galactosidase showed that after transplantation of fibroblast-like mesenchymal stem cells and embryonic fibroblasts, the transplanted cells remain viable on the surface and in the depth of the regenerating wounds throughout the entire observation period. The authors believe that the higher rate of burn wound regeneration with the use of fibroblast-like mesenchymal stem cells is due to the release of biologically active growth-stimulating factors by these cells during the maturation process.
Transplantation of auto- or allogeneic keratinocytes and allogeneic fibroblasts for the treatment of burn wounds has also been used in clinical practice. It should be noted that surgical treatment of children with extensive deep burns is a complex task due to the high traumatic nature and multiple surgical interventions, significant blood loss, and various reactions to the infusion media used. The main difficulties in performing skin plastic surgeries for extensive deep burns, with an area exceeding 40% of the body surface, are due to the severity of the condition of the victims and the lack of donor skin resources. The use of mesh transplants with a high perforation coefficient does not solve the problem, since the cells formed after perforation epithelialize very slowly, and the skin flaps themselves often lyse or dry out. Such coverings of burn wounds as xenoskin, cadaveric allografts, synthetic film coverings are not always effective enough, therefore new methods of covering burn surfaces with layers of cultured keratinocytes and fibroblasts are being developed. In particular, a method of covering burn surfaces with the help of cultured allofibroblasts has been proposed, which, when transplanted, have a pronounced stimulating effect on the proliferation of epidermocytes preserved in the wound in borderline burns, as well as keratinocytes in the septa of mesh transplants. The work of L. Budkevich and co-authors (2000) presents the results of using this method for treating burns in children. The study included 31 children with thermal trauma aged from 1 year to 14 years. In three children, the total area of burn wounds of grades IIIA-B - IV was 40%, in 25 - 50-70%, in another three - 71-85% of the body surface. Early surgical necrectomy was combined with transplantation of cultured allofibroblasts and autodermoplasty. The first stage of treatment involved excision of necrotic tissues, the second stage involved transplantation of cultured allofibroblasts on carrier films, and the third stage (48 hours after transplantation of cultured allofibroblasts) involved removal of the matrix and autodermoplasty with skin flaps with a perforation ratio of 1:4. Three patients admitted to the clinic with severe burn disease had cultured allofibroblasts transplanted onto granulating wounds. Transplantation of cultured allofibroblasts was performed once in 18 children, twice in 11 children, and three times in two patients. The area of the wound surface covered with cell culture ranged from 30 to 3500 cm2. The effectiveness of cultured allofibroblasts was assessed by the overall percentage of skin graft engraftment, burn healing times, and the number of fatalities from severe thermal trauma. Graft engraftment was complete in 86% of patients. Partial non-engraftment of skin grafts was noted in 14% of cases. Despite the treatment, six (19.3%) children died. The total area of skin damage in them ranged from 40 to 70% of the body surface.Transplantation of cultured allofibroblasts was not associated with mortality in burn injury in any patient.
Analyzing the treatment results, the authors note that previously deep thermal skin damage covering 35-40% of the body surface was considered incompatible with life (for younger children - up to 3 years old - deep burns covering 30% of the body surface are critical, for older children - over 40% of the body surface). When performing surgical necrectomy with transplantation of cultured allofibroblasts and subsequent autodermoplasty with skin flaps with a high perforation coefficient, IIIB - IV degree burns remain critical, but at present there are prospects for saving the lives of even such victims in many cases. Surgical necrectomy in combination with transplantation of cultured allofibroblasts and autodermoplasty in children with deep burns has proven to be especially effective in patients with widespread skin lesions with a shortage of donor sites. Active surgical tactics and transplantation of cultured allofibroblasts contribute to rapid stabilization of the general condition of such patients, a decrease in the number of infectious complications of burn disease, the creation of favorable conditions for the engraftment of transplants, a reduction in the time of restoration of lost skin and the duration of inpatient treatment, a decrease in the frequency of fatal outcomes in victims with extensive burns. Thus, transplantation of cultured allofibroblasts with subsequent autodermoplasty with skin flaps allows for recovery in children with severe burns, who were previously considered doomed.
It is generally accepted that the primary objective of treating burn disease is the most complete and rapid restoration of damaged skin to prevent toxic effects, infectious complications and dehydration. The results of using cultured cells largely depend on the readiness of the burn wound itself for transplantation. In cases of transplantation of cultured keratinocytes onto the wound surface after surgical necrectomy, an average of 55% (by area) of transplanted cells engraft, whereas with granulating wounds the engraftment rate decreases to 15%. Therefore, successful treatment of extensive deep skin burns requires, first of all, active surgical tactics. In the presence of burn wounds of IIIB-IV degree, the burn surface is immediately freed from necrotic tissue in order to reduce intoxication and reduce the number of complications of burn disease. The use of such tactics is the key to reducing the time from the moment of receiving a burn until the wounds close and the length of stay of patients with extensive burns in hospital, and also significantly reduces the number of fatal outcomes.
The first reports of successful use of cultured keratinocytes to cover burn surfaces appeared in the early 1980s. Subsequently, this manipulation was performed using layers of cultured keratinocytes, most often obtained from autocells, much less often from allokeratinocytes. However, the technology of autokeratinocytoplasty does not allow for the creation of a cell bank, while the time required to produce a keratinocyte transplant of sufficient area is long and amounts to 3-4 weeks. During this period, the risk of developing infectious and other complications of burn disease increases sharply, which significantly prolongs the total time of patients' stay in hospital. In addition, autokeratinocytes practically do not take root when transplanted onto granulating burn wounds, and the high cost of special growth media and biologically active stimulators of keratinocyte growth significantly limits their clinical use. Other biotechnological methods, such as collagenoplasty, transplantation of cryopreserved xenoskin, and the use of various biopolymer coatings increase the effectiveness of treating extensive superficial, but not deep burns. The method of covering the wound surface with cultured fibroblasts is fundamentally different in that fibroblasts, rather than keratinocytes, are used as the main component of the cultured cell layer.
The prerequisite for the development of the method was the data that pericytes surrounding small vessels are progenitor mesenchymal cells capable of transforming into fibroblasts that produce many growth factors and ensure wound healing due to a strong stimulating effect on the proliferation and adhesion of keratinocytes. The use of cultured fibroblasts to close wound surfaces immediately revealed a number of significant advantages of this method compared to the use of cultured keratinocytes. In particular, obtaining fibroblasts in culture does not require the use of special nutrient media and growth stimulants, which reduces the cost of the transplant by more than 10 times compared to the cost of obtaining keratinocytes. Fibroblasts are easily passivated, during which they partially lose surface histocompatibility antigens, which in turn opens up the possibility of using allogeneic cells for the manufacture of transplants and the creation of their banks. The time required to obtain transplants ready for use in a clinic is reduced from 3 weeks (for keratinocytes) to 1-2 days (for fibroblasts). A primary fibroblast culture can be obtained by culturing cells from skin fragments taken during autodermoplasty, and the cell seeding density for obtaining human fibroblast subcultures is only 20 x 10 3 per 1 cm 2.
In order to study the effect of fibroblasts and their regulatory proteins on the proliferation and differentiation of keratinocytes, a comparative analysis of the morphology and proliferation of keratinocytes on substrates of collagen types I and III, as well as fibronectin in a joint culture with human fibroblasts was performed. Human keratinocytes were isolated from skin fragments of patients with burns, taken during autodermoplasty. The keratinocyte seeding density was 50 x 103 cells per 1 cm2. The clinical efficacy of cultured fibroblast transplantation was assessed in 517 patients. All patients were divided into two groups: Group 1 - adult victims with burns of IIA, B - IV degree; Group 2 - children with deep burns of IIIB - IV degree. Evaluation of the dynamics of the structural and functional organization of monolayer culture fibroblasts taking into account the role of glycosaminoglycans, fibronectin and collagen in the regeneration processes allowed the authors to determine the 3rd day as the most favorable period for using fibroblast cultures to make transplants. A study of the effect of fibroblasts on the proliferation and differentiation of keratinocytes showed that in vitro fibroblasts have a pronounced stimulating effect, primarily on the processes of keratinocyte adhesion, increasing the number of attached cells and the rate of their fixation by more than 2 times. Stimulation of adhesion processes is accompanied by an increase in the intensity of DNA synthesis and the level of keratinocyte proliferation. In addition, it turned out that the presence of fibroblasts and the extracellular matrix formed by them is a necessary condition for the formation of the tonofibrillar apparatus of keratinocytes, intercellular connections and, ultimately, for the differentiation of keratinocytes and the formation of the basal membrane. In the treatment of children with deep burns, high clinical efficiency of transplantation of allofibroblast culture has been established, especially in the group of patients with extensive skin lesions in conditions of donor site deficiency. A comprehensive morphofunctional study has shown that transplant fibroblasts are characterized by active synthesis of DNA, as well as collagen, fibronectin and glycosaminoglycans, which are part of the extracellular matrix formed by cells. The authors point to a high percentage of engraftment of transplanted fibroblasts (up to 96%), a sharp reduction in the time of their receipt (within 24-48 hours instead of 2-3 weeks in the case of using keratinocytes), a significant acceleration of epithelialization of the burn surface, as well as a significant reduction in the cost (by 10 times) of the technology for growing a transplant from fibroblasts compared to keratinocyte transplantation. The use of transplantation of cultured allofibroblasts makes it possible to save the lives of children with critical burns - thermal damage to more than 50% of the body surface, which was previously considered incompatible with life. It should be noted thatthat with the transplantation of allogeneic embryonic fibroblasts, not only faster wound regeneration and convalescence of patients with varying degrees and areas of burns, but also a significant reduction in their mortality have also been convincingly proven.
Autologous fibroblasts are also used in such a complex area of plastic surgery as reconstructive correction of vocal cord injuries. Bovine collagen is usually used for this purpose, the duration of action of which is limited by its immunogenicity. Being a foreign protein, bovine collagen is sensitive to the recipient's collagenase and can cause immune reactions, to reduce the risk of which technologies for obtaining collagen preparations cross-linked with glutaraldehyde were developed. Their advantage lies in greater stability and lower immunogenicity, which has found practical application in eliminating defects and atrophy of the vocal cords. Injections of autologous collagen were first used in 1995. The technique ensured the preservation of the primary structure of autologous collagen fibers, including intramolecular enzymatically catalyzed cross-links. The fact is that natural collagen fibers are more resistant to destruction by proteases than reconstituted collagen, in which the telopeptides are cut. The integrity of telopeptides is important for the quaternary structure of collagen fibers and the formation of cross-links between adjacent collagen molecules. Unlike bovine collagen preparations, autologous collagen does not cause immune reactions in the recipient, but it is not effective enough as a replenishing agent. A stable correction can be achieved through local collagen production by transplantation of autologous fibroblasts. However, certain difficulties were identified during the study of the effectiveness of autologous fibroblast transplantation in the clinic. In the early period after fibroblast transplantation, the clinical effect was weaker compared to that after the introduction of bovine collagen. When culturing autologous fibroblasts, the possibility of transformation of normal fibroblasts into pathological ones, the so-called myofibroblasts, responsible for the development of fibrosis and scar formation, as evidenced by the contraction of the collagen gel caused by the specific interaction of fibroblasts and collagen fibrils, cannot be ruled out. In addition, after serial passaging in vitro, fibroblasts lose the ability to synthesize extracellular matrix proteins.
However, a method for culturing autologous human fibroblasts has now been experimentally developed that eliminates the above-mentioned shortcomings and does not result in oncogenic transformation of normal fibroblasts. Autologous fibroblasts obtained using this method are used to restore defects in soft facial tissues. In a study by G. Keller et al. (2000), 20 patients aged 37 to 61 years with wrinkles and atrophic scars were treated. Skin biopsies (4 mm) from the retroauricular region were transported to the laboratory in sterile test tubes containing 10 ml of culture medium (Eagle's medium with antibiotic, mycoseptic, pyruvate, and fetal calf serum). The material was placed inside 3-5 culture dishes 60 mm in diameter and incubated in a thermostat with an atmosphere containing 5% CO2. After 1 week, the cells were removed from the dishes by trypsinization and placed in 25 cm2 vials. The cells were injected into patients in an amount of 4 x 107. A significant and persistent clinical effect was observed in patients during the correction of nasolabial folds, as well as in patients with scars 7 and 12 months after the third transplantation of autologous fibroblasts. According to flow cytometry, the cultured fibroblasts produced a large amount of type I collagen. In vitro studies have shown normal contractility of the injected fibroblasts. Two months after the subcutaneous administration of cultured fibroblasts at a dose of 4 x 107 cells, no tumors were detected in nude mice. The injected fibroblasts did not cause scarring or diffuse fibrosis in patients. According to the author, the engrafted autologous fibroblasts are capable of constantly producing collagen, which will provide a cosmetic rejuvenation effect. At the same time, since the lifespan of differentiated cells is limited, fibroblasts taken from a young patient are more effective than those obtained from elderly people. In the future, it is assumed that it will be possible to cryopreserve a culture of fibroblasts taken from a young donor in order to later transplant his own young cells to an elderly patient. In conclusion, it is not entirely correct to conclude that autologous fibroblasts, provided that they are functionally preserved, are an ideal means of correcting defects of soft tissues of the face. At the same time, the author himself notes that some problematic situations related to the use of the autologous fibroblast-collagen system arose during the study. The clinical effect was often weaker than when using bovine collagen, which caused disappointment in patients.
In general, the literature data on the prospects for the clinical use of mesenchymal stem cells look quite optimistic. Attempts are being made to use autologous bone marrow multipotent mesenchymal progenitor cells to treat degenerative joint lesions. The first clinical trials of the use of cultured mesenchymal progenitor cells in the treatment of complex bone fractures are being conducted. Auto- and allogeneic mesenchymal bone marrow stromal cells are used to create cartilage tissue for transplantation in the correction of articular cartilage defects due to trauma or autoimmune lesions. Methods are being developed for the clinical use of multipotent mesenchymal progenitor cells to eliminate bone defects in children with a severe form of incomplete osteogenesis caused by mutations in the type I collagen gene. After myeloablation, recipient children are transplanted with bone marrow from HLA-compatible healthy donors, since unfractionated bone marrow can contain a sufficient number of mesenchymal stem cells to compensate for a severe bone defect. After transplantation of allogeneic bone marrow, such children have shown positive histological changes in trabecular bones, an increase in the growth rate, and a decrease in the incidence of bone fractures. In some cases, a positive clinical result is achieved by transplanting closely related allogeneic bone marrow and osteoblasts. MSC transplantation is also used to treat congenital bone fragility caused by an imbalance of osteoblasts and osteoclasts in bone tissue. In this case, restoration of bone formation is achieved through chimerization of the pool of stem and progenitor stromal cells in the bone tissue of patients.
Improvement of methods of genetic modification of donor mesenchymal stem cells for the purpose of correction of genetic defects of stromal tissues is continued. It is assumed that in the nearest future mesenchymal progenitor cells will be used in neurology for targeted chimerization of brain cells and creation of healthy pool of cells capable of generating deficient enzyme or factor responsible for clinical manifestations of disease. Transplantation of mesenchymal stem cells can be used for restoration of bone marrow stroma in cancer patients after radio- and chemotherapy, and in combination with bone marrow cells - for restoration of hematopoiesis. Development of replacement therapy aimed at elimination of defects of musculoskeletal system with the help of MSCs is promoted by engineering developments in the field of designing matrix biomaterials or biomimetics forming frameworks populated by progeny of mesenchymal stem cells.
Sources of mesenchymal stem cells
The main source of mesenchymal stem cells is bone marrow, whose hematopoietic stem cells in the body of mammals constantly differentiate into blood and immune system cells, while mesenchymal stem cells are represented by a small population of fibroblast-like cells of the bone marrow stroma and contribute to the preservation of the undifferentiated state of hematopoietic stem cells. Under certain conditions, mesenchymal stem cells differentiate into cartilage and bone tissue cells. When seeded on a culture medium under low-density planting conditions, mononuclear stromal cells of the bone marrow form colonies of adhesive cells, which are, in fact, fibroblast-like multipotent mesenchymal progenitor cells. Some authors believe that uncommitted mesenchymal stem cells are deposited in the bone marrow, which, due to their ability to self-renew and high differentiation potential, provide all tissues of the body with mesenchymal precursors of stromal elements throughout the life of the mammalian organism.
In the bone marrow, stromal cellular elements form a network filling the space between the sinusoids and bone tissue. The content of dormant MSCs in the bone marrow of an adult is comparable to the amount of hematopoietic stem cells and does not exceed 0.01-0.001%. Mesenchymal stem cells isolated from bone marrow and not subjected to cultivation are devoid of adhesion molecules. Such MSCs do not express CD34, ICAM, VCAM, collagen types I and III, CD44 and CD29. Consequently, in vitro, it is not mesenchymal stem cells that are fixed on the culture substrate, but more advanced progenitor derivatives of mesenchymal stem cells that have already formed the components of the cytoskeleton and the receptor apparatus of cell adhesion molecules. Stromal cells with the CD34 phenotype are found even in peripheral blood, although in the bone marrow there are significantly fewer of them than CD34-positive mononuclear cells. CD34 cells isolated from the blood and transferred to culture attach to the substrate and form colonies of fibroblast-like cells.
It is known that in the embryonic period the stromal basis of all organs and tissues of mammals and humans arises from a common pool of mesenchymal stem cells before and at the stage of organogenesis. Therefore, it is believed that in a mature organism the majority of mesenchymal stem cells should be in the connective and bone tissue. It has been established that the main part of the cellular elements of the stroma of loose connective and bone tissue is represented by committed progenitor cells, which, however, retain the ability to proliferate and form clones in vitro. When such cells are introduced into the general bloodstream, more than 20% of mesenchymal progenitor cells are implanted among the stromal elements of the hematopoietic tissue and parenchymatous organs.
A potential source of mesenchymal stem cells is adipose tissue, among the stem cells of which adipocyte precursors committed to varying degrees have been identified. The least mature progenitor elements of adipose tissue are stromal-vascular cells, which, like multipotent mesenchymal precursor cells of bone marrow, are capable of differentiating into adipocytes under the influence of glucocorticoids, insulin-like growth factor, and insulin. In culture, stromal-vascular cells differentiate into adipocytes and chondrocytes, and in adipose tissue of bone marrow origin there are cells that form adipocytes and osteoblasts.
Stromal stem cells have also been found in muscles. In the primary culture of cells isolated from human skeletal muscle, stellate cells and multinucleated myotubes are detected. In the presence of horse serum, stellate cells proliferate in vitro without signs of cytodifferentiation, and after the addition of dexamethasone to the nutrient medium, their differentiation is characterized by the appearance of cellular elements with the phenotype of skeletal and smooth muscle cells, bone, cartilage, and adipose tissue. Therefore, both committed and uncommitted multipotent mesenchymal progenitor cells are present in human muscle tissue. It has been shown that the population of progenitor cells present in skeletal muscle originates from uncommitted multipotent mesenchymal progenitor cells of the bone marrow and differs from myogenic satellite cells.
Adhesive stellate cells corresponding to multipotent mesenchymal progenitor cells in differentiation potential were also found in the myocardium of newborn rats, since under the influence of dexamethasone they differentiate into adipocytes, osteoblasts, chondrocytes, smooth muscle cells, skeletal muscle myotubes and cardiomyocytes. It was shown that vascular smooth muscle cells (pericytes) are derivatives of undifferentiated perivascular multipotent mesenchymal progenitor cells. In culture, perivascular mesenchymal stem cells express smooth muscle a-actin and platelet-derived growth factor receptor and are capable of differentiating at least into smooth muscle cells.
A special place, from the point of view of stem reserves, is occupied by cartilaginous tissue, the extremely low reparative potential of which is believed to be due to a deficiency of multipotent mesenchymal progenitor cells or differentiation and growth factors. It is assumed that multipotent mesenchymal progenitor cells precommitted to chondro- and osteogenesis enter cartilaginous tissue from other tissue sources.
The tissue origin and conditions of commitment of mesenchymal progenitor cells in tendons have also not been established. Experimental observations indicate that in the early postnatal period, rabbit Achilles tendon cells in primary cultures and at the first passage retain the expression of type I collagen and decorin, but with further cultivation they lose the differentiation markers of tenocytes.
It should be noted that the answer to the question of whether multipotent mesenchymal progenitor cells localized in various tissues are actually constantly present in their stroma, or whether the tissue pool of mesenchymal stem cells is replenished by the migration of bone marrow stromal stem cells, has not yet been received.
In addition to bone marrow and other mesenchymal tissue zones of an adult organism, cord blood can be another source of MSCs. It has been shown that umbilical cord vein blood contains cells that have similar morphological and antigenic characteristics with multipotent mesenchymal progenitor cells, are capable of adhesion and are not inferior to multipotent mesenchymal progenitor cells of bone marrow origin in differentiation potential. In cultures of mesenchymal stem cells of umbilical cord blood, 5 to 10% of uncommitted multipotent mesenchymal progenitor cells were found. It turned out that their number in cord blood is inversely proportional to the gestational age, which indirectly indicates the migration of multipotent mesenchymal progenitor cells to various tissues during fetal development. The first information has appeared on the clinical use of mesenchymal stem cells isolated from umbilical cord blood, as well as those obtained from embryonic biomaterial, which is based on the known ability of fetal stem cells to integrate, engraft, and function in the organs and tissue systems of adult recipients.
Search for new sources of mesenchymal stem cells
The use of mesenchymal stem cells of embryonic origin, as well as other fetal cells, creates a number of ethical, legal, judicial and legislative problems. Therefore, the search for extraembryonic donor cell material continues. An attempt at clinical use of human skin fibroblasts was unsuccessful, which was predetermined not only by the high financial capacity of the technology, but also by the rapid differentiation of fibroblasts into fibrocytes, which have a significantly lower proliferation potential and produce a limited number of growth factors. Further progress in the study of the biology of MSCs and multipotent mesenchymal progenitor cells of bone marrow allowed us to develop a strategy for the clinical use of autologous mesenchymal stem cells. The technology of their isolation, cultivation, ex vivo reproduction and targeted differentiation required, first of all, the study of the spectrum of molecular markers of MSCs. Their analysis showed that primary cultures of human bone tissue contain several types of multipotent mesenchymal progenitor cells. The proosteoblast phenotype was detected in cells expressing the marker of stromal progenitor cells STRO-1, but not carrying the osteoblast marker - alkaline phosphatase. Such cells are characterized by a low ability to form mineralized bone matrix, as well as the absence of osteopontin and parathyroid hormone receptor expression. Derivatives of STRO-1-positive cells that do not express alkaline phosphatase are represented by intermediately and completely differentiated osteoblasts. It was found that cellular elements of cloned lines of STRO-1-positive human trabecular bone cells are capable of differentiating into mature osteocytes and adipocytes. The direction of differentiation of these cells depends on the effect of polyunsaturated fatty acids, proinflammatory cytokines - IL-1b and tumor necrosis factor a (TNF-a), as well as anti-inflammatory and immunosuppressive TGF-b.
It was later found that multipotent mesenchymal progenitor cells lack a specific phenotype inherent only to them, but express a complex of markers characteristic of mesenchymal, endothelial, epithelial and muscle cells in the absence of expression of immunophenotypic antigens of hematopoietic cells - CD45, CD34 and CD14. In addition, mesenchymal stem cells constitutively and inducibly produce hematopoietic and non-hematopoietic growth factors, interleukins and chemokines, and receptors for some cytokines and growth factors are expressed on multipotent mesenchymal progenitor cells. Dormant, or resting, cells with an immunophenotype almost identical to the antigen profile of 5-fluorouracil-untreated multipotent mesenchymal progenitor cells have been found among the cells of the stromal matrix of the human body - both cells express CD117, which marks “adult” stem cells.
Thus, a cell marker unique to mesenchymal stem cells has not yet been identified. It is assumed that quiescent cells represent a population of uncommitted multipotent mesenchymal progenitor cells, since they do not express markers of cells committed to osteo- (Cbfa-1) or adipogenesis (PPAR-y-2). Prolonged exposure of slowly proliferating quiescent cells to fetal bovine serum leads to the formation of terminally differentiating committed progenitors characterized by rapid growth. Clonal expansion of such mesenchymal stem cells is supported by FGF2. It seems that the genome of stromal stem cells is quite tightly “closed”. There are reports of the absence of spontaneous differentiation in MSCs - without special conditions for commitment, they do not transform even into cells of the mesenchymal lineage.
To study the population structure of mesenchymal stem cell derivatives, a search for differentiation marker proteins is carried out on stromal cell lines and in primary cultures. In vitro clonal analysis of bone marrow colony-forming cells has shown that EGF increases the average colony size and decreases clonal expression of alkaline phosphatase when applied to primary cultures, while the addition of hydrocortisone activates the expression of alkaline phosphatase, which is a marker of the osteogenic direction of MSC differentiation. Monoclonal antibodies to STRO-1 made it possible to separate and study the population of STRO-1-positive adhesive cells in a heterogeneous system of Dexter cultures. A spectrum of cytokines has been determined that regulate not only the proliferation and differentiation of hematopoietic and lymphoid cells, but also participate in the formation, formation and resorption of skeletal tissues through para-, auto- and endocrine mechanisms. Receptor-mediated release of such secondary messengers as cAMP, diacylglycerol, inositol triphosphate and Ca2+ is also used for marker analysis of various categories of stromal tissue cells expressing the corresponding receptors. The use of monoclonal antibodies as markers made it possible to establish the belonging of reticular cells of the stroma of lymphoid organs to the T- and B-dependent zones.
For some time, scientific debates continued around the question of the possibility of MSC origin from a hematopoietic stem cell. Indeed, when bone marrow cell suspensions are explanted into monolayer cultures, discrete colonies of fibroblasts grow in them. However, it was shown that the presence of precursors of fibroblast colonies and various sprouts of hematopoietic tissue differentiation in the bone marrow is not evidence of their common origin from a hematopoietic stem cell. Using discriminant analysis of bone marrow stem cells, it was established that the microenvironment during heterotopic bone marrow transplantation is not transferred by hematopoietic cells, which proves the existence of a population of MSCs in the bone marrow that is histogenetically independent of hematopoietic cells.
In addition, the selective cloning method made it possible to identify a new category of stromal progenitor cells in monolayer cultures of bone marrow cells, determine their numbers, and study their properties, proliferative and differentiating potentials. It turned out that stromal fibroblast-like cells proliferate in vitro and form diploid colonies, which, when transplanted back into the body, provide for the formation of new hematopoietic organs. The results of the study of individual clones indicate that among the stromal progenitor cells there is a population of cells that, by their proliferative and differentiating potential, can claim the role of stem cells of stromal tissue, histogenetically independent of hematopoietic stem cells. The cells of this population are characterized by self-sustaining growth and differentiate into progenitor cell elements of bone, cartilage and reticular tissue of the bone marrow.
Of great interest are the results of the studies by R. Chailakhyan and co-authors (1997-2001), who cultivated bone marrow stromal progenitor cells from rabbits, guinea pigs, and mice on the a-MEM nutrient medium with the addition of fetal calf serum. The authors performed explantation with an initial density of 2-4 x 103 bone marrow cells per 1 cm2. Homologous or heterologous radiation-inactivated bone marrow cells were used as a feeder in a dose that retained the feeder effect but completely blocked their proliferation. Two-week-old primary discrete colonies of fibroblasts were trypsinized to obtain monoclonal strains. Evidence of the clonal origin of the colonies was obtained using a chromosomal marker in mixed bone marrow cultures of male and female guinea pigs, time-lapse photography of live cultures, and in mixed cultures of syngeneic bone marrow of CBA and CBAT6T6 mice. Transplantation of a suspension of freshly isolated bone marrow cells or in vitro grown stromal fibroblasts under the kidney capsule was performed in ivalon or gelatin porous scaffolds, as well as inactivated rabbit spongy bone matrix. For transplantation of clones in a bone sheath, guinea pig femurs were cleared of soft tissue and periosteum, epiphyses were trimmed, and bone marrow was thoroughly washed out. The bone was cut into fragments (3-5 mm), dried, and irradiated at a dose of 60 Gy. Individual colonies of fibroblasts were placed into bone sheaths and implanted intramuscularly. For intraperitoneal transplantation of stromal fibroblasts grown in vitro, diffusion chambers of types A (V=0.015 cm3, h=0.1 mm) and O (V=0.15 cm3, h=2 mm) were used.
When studying the growth dynamics of clonal strains, R. Chailakhyan et al. (2001) found that individual cells forming fibroblast colonies, as well as their descendants, have enormous proliferative potential. By the 10th passage, the number of fibroblasts in some strains was 1.2-7.2 x 10 9 cells. During their development, they performed up to 31-34 cell doublings. In this case, heterotopic transplantation of bone marrow-derived strains formed by stromal precursors of several dozen clones resulted in the transfer of the bone marrow microenvironment and the formation of a new hematopoietic organ in the transplantation zone. The authors posed the question of whether individual clones are capable of transferring the bone marrow microenvironment of stromal cells or whether cooperation of several different clonogenic stromal precursors is required for this? And if individual clones are capable of transferring the microenvironment, will it be complete for all three hematopoietic sprouts, or do different clones provide the formation of the microenvironment for different hematopoietic sprouts? To solve these issues, a technology was developed for culturing stromal progenitor cells on a collagen gel, allowing the grown colonies of fibroblasts to be removed from the surface for subsequent heterotopic transplantation. Individual clones of stromal fibroblasts grown from bone marrow cells of CBA mice and guinea pigs were excised together with a fragment of the gel coating and heterotopically transplanted - under the kidney capsule of syngeneic mice or into the abdominal muscle of autologous guinea pigs. When transplanted into the muscle, the colonies on the gel were placed in bone sheaths.
The authors found that 50-90 days after transplantation of bone marrow fibroblast colonies, development of bone or bone and hematopoietic tissue was observed in the transplantation zone in 20% of cases. In 5% of recipient animals, the formed foci of bone tissue contained a cavity filled with bone marrow. Inside the bone cylinders, such foci had a rounded shape and a capsule built of bone tissue with osteocytes and a well-developed osteoblastic layer. The bone marrow cavity contained reticular tissue with myeloid and erythroid cells, the proportional relationship of which did not differ from that in normal bone marrow. In the kidney, the transplant was a typical bone marrow organ formed during transplantation of native bone marrow, with the bone capsule covering the bone marrow cavity only from the side of the renal capsule. The hematopoietic tissue included myeloid, erythroid and megakaryocytic elements. The stroma of the bone marrow cavity had a well-developed sinus system and contained typical fat cells. At the same time, bone tissue without signs of hematopoiesis was found in the transplantation zone of some colonies under the kidney capsule. The study of the proliferative and differentiating potentials of individual clones was continued on monoclonal bone marrow strains of rabbits, the cells of which were resuspended in a nutrient medium and in a separate ivalon sponge with a mass of 1-2 mg were transplanted under the renal capsule of a rabbit-bone marrow donor. Cells of 21 monoclonal strains were subjected to such autotransplantation. The results were taken into account after 2-3 months. The authors found that in 14% of cases, the transplanted monoclonal strains formed a bone marrow organ consisting of bone tissue and a bone marrow cavity filled with hematopoietic cells. In 33% of cases, the transplanted strains formed a compact bone of varying size with osteocytes immured in the cavities and a developed osteoblastic layer. In some cases, reticular tissue without bone or hematopoietic elements developed in the sponges with transplanted clones. Sometimes, reticular stroma with a well-developed network of sinusoids was formed, but not populated with hematopoietic cells. Thus, the obtained results were similar to the data obtained during clone transplantation on collagen gel. However, if transplantation of clones grown on a substrate resulted in the formation of bone marrow tissue in 5% of cases, bone tissue in 15% and reticular tissue in 80% of cases, then with transplantation of monoclonal strains, the formation of bone marrow elements was observed in 14% of cases, bone tissue in 53% and reticular tissue in 53% of cases. According to the authors, this indicates that the conditions for the implementation of proliferative and differentiating potential of stromal fibroblasts during transplantation on porous scaffolds were more optimal than during their transplantation in bone sheaths and on a collagen substrate. It is possible thatthat the use of more advanced methods of culturing and reverse transplantation of clones can improve the conditions for the realization of their differentiation potential by clones and change these ratios. One way or another, but the main significance of the conducted studies is that some clones of stromal cells are capable of forming bone tissue and simultaneously providing a stromal hematopoietic microenvironment for three sprouts of bone marrow hematopoiesis at once: erythroid, myeloid and megakaryocytic, creating quite large platforms of hematopoietic tissue and some bone mass.
The authors then addressed the issue of the ability of individual clonogenic stromal progenitor cells to undergo these types of cell differentiation in a closed system of diffusion chambers. In addition, it was necessary to determine whether individual clones possess polypotency or whether the manifestation of differentiation potential requires cooperative interaction of several clones with a fixed cytodifferentiation trait, the different ratios of which determine the preferential formation of bone, reticular, or cartilaginous tissue. By combining two methodological approaches - obtaining monoclonal strains of bone marrow stromal progenitor cells and transplanting them into diffusion chambers - R. Chailakhyan and co-authors (2001) obtained results that allowed them to come closer to understanding the structural organization of bone marrow stroma. Transplantation of monoclonal strains of stromal progenitor cells into O-type chambers resulted in the formation of both bone and cartilage tissue, indicating the ability of the descendants of a single stromal colony-forming cell to simultaneously form bone and cartilage tissue. The assumption that bone and cartilage tissue originate from a common stromal progenitor cell has been repeatedly put forward. However, this hypothesis had no correct experimental confirmation. The formation of bone and cartilage in diffusion chambers was the necessary proof of the existence of a common progenitor cell for these two types of tissue among bone marrow stromal stem cells.
Then 29 clonal strains of the second-third passages obtained from primary cultures of rabbit bone marrow were placed in diffusion chambers and implanted intraperitoneally into homologous animals. The studies showed that 45% of bone marrow monoclonal strains possess osteogenic potential. Nine chambers contained exclusively reticular tissue, but it was present together with bone and cartilage tissue in 13 more chambers, which constituted 76% of all strains. In type O chambers, where differentiation of both bone and cartilage tissue was possible, 16 strains were studied. In four chambers (25%) both bone and cartilage tissue were formed. It should be noted once again that in the studies of R. Chailakhyan et al. (2001), individual progenitor cells underwent 31 to 34 doublings within a cell strain, and their progeny comprised 0.9-2.0 x 10 9 cells. The number of mitoses that progenitor cells of polyclonal strains underwent was virtually identical to that of monoclonal strains. The rate of development of polyclonal strains, especially in the first phase of their formation, depended to a significant extent on the number of colonies used to initiate the strains. Diploid strains of human embryonic fibroblasts (WI-38), when recloned at the 12-15th doubling levels, also formed colonies that differed in diameter and cell content. Large colonies containing more than 103 cells constituted only 5-10%. With an increase in the number of divisions, the percentage of large colonies decreased. Mono- and polyclonal strains of bone marrow stromal fibroblasts retained a diploid set of chromosomes after 20 or more doublings, and the tendency of their development was comparable with the dynamics of development of diploid strains of embryonic fibroblasts. Analysis of the differentiation potential of individual bone marrow stromal progenitor cells, carried out by transplanting monoclonal strains into diffusion chambers, showed that half of them were osteogenic. Large colonies accounted for 10% of their total number. Consequently, the number of osteogenic colony-forming cells corresponded to approximately 5% of their total population. The total mass of osteogenic progenitor cells identified by the authors included cells capable of simultaneously forming bone and cartilage tissue. Moreover, it was established for the first time that these two types of tissue in an adult organism have a common progenitor cell: 25% of the tested clones were created by such cells, and their number among the total population of progenitor cells was at least 2.5%.
Thus, heterotopic transplantation of individual clones of bone marrow fibroblasts has revealed new aspects of the structural organization of the population of mesenchymal progenitor cells. Stromal progenitor cells have been found that are capable of transferring a specific microenvironment for all hematopoietic sprouts at once, the number of which among the large clones studied in different models ranges from 5 to 15% (0.5-1.5% of the total number of progenitor cells detected). Along with clones that transfer the complete bone marrow microenvironment, there are progenitor cells determined only to osteogenesis, which, when transferred in an open system, form bone tissue that does not support the development of hematopoiesis. Their number from the total number of progenitor cells is 1.5-3%. Some of these cells are capable of forming bone tissue with a limited period of self-maintenance. Consequently, the population of stromal progenitor cells is heterogeneous in its differentiation potential. Among them, there is a category of cells that claim to be stromal stem cells, capable of differentiating in all three directions characteristic of bone marrow stromal tissue, forming bone, cartilage, and reticular tissue. The presented data allow us to hope that, using various cell markers, it will be possible to determine the contribution of each type of stromal cells to the organization of a specific microenvironment and support of hematopoiesis in Dexter cultures.
Features of mesenchymal stem cells
In recent years, it has been established that in stationary bone marrow cultures, multipotent mesenchymal progenitor cells are represented by a limited population of small agranular cells (RS-1 cells) characterized by a low colony-forming capacity and the absence of expression of the Ki-67 antigen specific for proliferating cells. The antigenic parameters of dormant RS-1 cells differ from the spectrum of antigens of rapidly proliferating committed stromal progenitor cells. It has been established that a high proliferation rate of committed progenitor cells is observed only in the presence of RS-1 cells. In turn, RS-1 cells increase their growth rate under the influence of factors secreted by the most mature derivatives of multipotent mesenchymal progenitor cells. It seems that RS-1 cells are a subclass of uncommitted MSCs capable of recycling. In vitro, 5-fluorouracil-resistant bone marrow stromal progenitor cells are characterized by low RNA content and high expression of the ornithine decarboxylase gene, a marker of non-proliferating cells.
Intensive proliferation of stromal progenitor cells begins after their fixation on the substrate. In this case, the marker profile of poorly differentiated cells is expressed: SH2 (TGF-(3) receptor), SH3 (signaling protein domain), collagen types I and III, fibronectin, adhesion receptors VCAM-1 (CD106) and ICAM (CD54), cadherin-11, CD44, CD71 (transferrin receptor), CD90, CD120a and CD124, but without the expression of characteristic markers of hematopoietic stem cells (CD34, CD14, CD45). Clonal growth makes it possible to repeatedly passage mesenchymal stem cells with the formation of numerous genetically homogeneous stromal progenitor pluripotent cells in culture. After 2-3 passages, their number reaches 50-300 million. In a culture of sufficient density, after proliferation has stopped, stromal progenitor cells, unlike hematopoietic tissue fibroblasts, differentiate into adipocytes, myocytes, cartilage and bone cells. A combination of three regulatory differentiation signals, including 1-methyl-isobutylxanthine (an inducer of intracellular cAMP formation), dexamethasone (an inhibitor of phospholipases A and C) and indomethacin (an inhibitor of cyclooxygenase, which also reduces the activity of thromboxane synthase), converts up to 95% of progenitor mesenchymal cells into adipocytes. The formation of adipocytes from immature stromal elements is confirmed by the expression of the lipoprotein lipase gene, histochemical detection of apolipoproteins and peroxisomal receptors. Cells of the same clone under the influence of TGF-b in a serum-free medium create a homogeneous population of chondrocytes. The multilayer cell culture of this cartilaginous tissue is characterized by a developed intercellular matrix consisting of proteoglycan and type II collagen. In a nutrient medium with 10% The effect of a differentiation signal complex consisting of b-glycerophosphate (an inorganic phosphate donor), ascorbic acid and dexamethasone in the same culture of stromal progenitor cells leads to the formation of cellular aggregates. In such cells, a progressive increase in alkaline phosphatase activity and osteopontin levels is observed, indicating the formation of bone tissue, the mineralization of the cells of which is confirmed by a progressive increase in intracellular calcium content.
According to some data, the ability of mesenchymal stem cells to unlimited division and reproduction of various types of cells of the mesenchymal differentiation line is combined with a high degree of plasticity. When introduced into the ventricles or white matter of the brain, mesenchymal stem cells migrate to the parenchyma of the nervous tissue and differentiate into derivatives of the glial or neuronal cell line. In addition, there is information on the transdifferentiation of MSCs into hematopoietic stem cells both in vitro and in vivo. A more in-depth analysis in some studies has determined an exceptionally high plasticity of MSCs, which is manifested in their ability to differentiate into astrocytes, oligodendrocytes, neurons, cardiomyocytes, smooth muscle cells and skeletal muscle cells. A number of studies on the transdifferentiation potential of MSCs in vitro and in vivo have established that multipotent mesenchymal progenitor cells of bone marrow origin terminally differentiate into cell lines that form bone, cartilage, muscle, nerve and adipose tissue, as well as tendons and stroma that support hematopoiesis.
However, other studies failed to reveal any signs of restriction of pluripotency of the mesenchymal stem cell genome and progenitor populations of stromal cells, although more than 200 clones of MSCs isolated from one primary culture were studied to test possible pluripotency of stromal cells. The overwhelming majority of clones in vitro retained the ability to differentiate in osteogenic, chondrogenic, and adipogenic directions. When excluding the probability of recipient cell migration by transplanting mesenchymal stem cells under the kidney capsule or in diffusion chambers, it turned out that stromal progenitor cells in situ retain a heterogeneous phenotype, which indicates either the absence of restriction factors in the transplantation zone or the absence of MSC pluripotency as such. At the same time, the existence of a rare type of somatic pluripotent stem cells, which are common precursors of all adult stem cells, is allowed.
The multi-, but not pluripotency, of true mesenchymal stem cells, which constitute a very small proportion of bone marrow cells and are capable of proliferating under certain conditions during in vitro cultivation without differentiating, is evidenced by their induced commitment to bone, cartilage, adipose, muscle tissue cells, as well as tenocytes and stromal elements that support hematopoiesis. As a rule, prolonged exposure to a culture medium with fetal calf serum provokes the release of MSCs into committed stromal progenitor cells, the progeny of which undergo spontaneous terminal differentiation. In vitro, it is possible to achieve targeted formation of osteoblasts by adding dexamethasone, ß-glycerophosphate, and ascorbic acid to the conditioning medium, while a combination of dexamethasone and insulin differentiation signals induces the formation of adipocytes.
It has been established that before entering the stage of terminal differentiation, bone marrow MSCs initially differentiate into fibroblast-like mesenchymal stem cells under certain culture conditions. Derivatives of these cells in vivo participate in the formation of bones, cartilage, tendons, adipose and muscle tissue, as well as the stroma that supports hematopoiesis. Many authors understand the term “multipotent mesenchymal progenitor cells” to mean both MSCs themselves and committed stromal progenitor cells of bone marrow and mesenchymal tissues. Clonal analysis of multipotent mesenchymal progenitor cells of bone marrow origin showed that slightly more than one third of all clones differentiate into osteo-, chondro- and adipocytes, while the cells of the remaining clones have only osteogenic potential and form only chondro- and osteocytes. A clone of multipotent mesenchymal progenitor cells such as BMC-9, under appropriate microenvironmental conditions, differentiates into cells with the phenotype and functional characteristics of not only osteoblasts, chondrocytes, and adipocytes, but also stromal cells that support hematopoiesis. A clone of RCJ3.1 cells isolated from rat fetal bone marrow differentiates into mesenchymal cells of various phenotypes. Under the combined action of ascorbic acid, b-glycerophosphate, and dexamethasone, the cellular elements of this clone first form multinuclear myocytes, and then, sequentially, adipocytes, chondrocytes, and islets of mineralized bone tissue. The population of granular cells from the periosteum of rat fetuses corresponds to uncommitted multipotent mesenchymal progenitor cells, since it is characterized by a low proliferation rate, does not express differentiation markers, and under culture conditions differentiates to form chondro-, osteo-, and adipocytes, as well as smooth muscle cells.
Thus, it should be recognized that the question of the pluri- or multipotency of the genome of mesenchymal stem cells remains open, which, accordingly, also affects the ideas about the differentiation potential of stromal progenitor cells, which has also not been definitively established.
An experimentally proven and important characteristic of mesenchymal stem cells is their ability to leave the tissue niche and circulate in the general bloodstream. To activate the genetic differentiation program, such circulating stem cells must enter the appropriate microenvironment. It has been shown that with systematic introduction of MSCs into the bloodstream of recipient animals, immature cells are implanted in various organs and tissues, then differentiating into blood cells, myocytes, adipocytes, chondrocytes, and fibroblasts. Consequently, in local tissue zones, signal-regulatory interactions occur between uncommitted and committed stromal progenitor cells, as well as between them and the surrounding mature cells. It is assumed that differentiation is induced by paracrine regulatory factors of mesenchymal and non-mesenchymal origin (growth factors, eicosanoids, extracellular matrix molecules), which provide spatial and temporal connections in the microenvironment of multipotent mesenchymal progenitor cells. Therefore, local damage to mesenchymal tissue should lead to the formation of zones of the microenvironment of multipotent mesenchymal progenitor cells that are qualitatively different from the complex of regulatory signals of intact tissues, in which physiological rather than reparative regeneration processes occur. This difference is extremely important in terms of specialization of the cellular phenotype in the normal and damage-induced microenvironment.
According to the concepts, it is here that the mechanisms of the fundamental difference between the two known processes - physiological regeneration and inflammatory proliferation - are embedded. The first of them ends with the restoration of the specialized cellular composition of the tissue and its function, while the result of the implementation of the proliferation process is the formation of mature connective tissue elements and the loss of function of the damaged tissue zone. Thus, to develop optimal programs for the use of multipotent mesenchymal progenitor cells in regenerative-plastic medicine, a thorough study of the features of the impact of microenvironmental factors on the differentiation of MSCs is necessary.
Dependence of the structure of the stem cell compartment on cellular para- and autocrine regulators, the expression of which is modulated by external signals, is beyond doubt. Among the functions of regulatory factors, the most important are control of asymmetric division of MSCs and expression of genes determining the stages of commitment and the number of cell divisions. External signals, on which further development of MSCs depends, are provided by their microenvironment. In an immature state, MSCs proliferate for a long time, while maintaining the ability to differentiate into lines of adipocytes, myofibroblasts, hematogenous tissue stroma, cartilage and bone cells. It has been established that a limited population of CD34-negative stromal cellular elements circulating in the blood returns from the general bloodstream to the bone marrow stroma, where it is transformed into lines of CD34-positive hematopoietic stem cells. These observations suggest that recirculation of progenitor mesenchymal cells in the bloodstream maintains tissue balance of stromal stem cells in different organs by mobilizing a common pool of immature stromal elements of the bone marrow. Differentiation of MSCs into cells with multiple mesenchymal phenotypes and their participation in regeneration or repair of bone, cartilage, adipose tissue and tendons in vivo has been proven using adoptive transfer models in experimental animals. According to other authors, distant migration of MSCs along the vascular bed is combined with short-distance or local movement of multipotent mesenchymal progenitor cells within the tissue during cartilage repair, muscle regeneration and other restorative processes.
Local stem reserves of the stromal tissue base act as a source of cells in the processes of physiological tissue regeneration and are replenished by distant transport of MSCs as stromal-tissue stem resources are consumed. However, in conditions of the need for emergency mobilization of reparative cellular potential, for example, in case of polytrauma, the entire echelon of MSCs takes part in the processes of reparative regeneration, and mesenchymal progenitor cells of the bone marrow are recruited to the periphery through the general blood flow.
Mesenchymal stem cell transplantation
Certain parallels can be traced between the processes of physiological tissue regeneration and their formation during intrauterine development. In human and mammalian embryogenesis, the formation of various types of specialized cells occurs from the ecto-, meso-, and endodermal pool of germ layers, but with the obligatory participation of the mesenchyme. The loose cellular network of embryonic mesenchymal tissue performs numerous regulatory, metabolic, framework, and morphogenetic functions. The laying of provisional organs occurs only after the condensation of the mesenchyme due to the clonogenic growth of progenitor cells, which generate the primary morphogenetic signals of organogenesis. Stromal derivatives of the embryonic mesenchyme create the cellular framework of provisional organs and form the basis for their future energy-plastic supply due to the growth of primary blood and lymphatic vessels. In other words, the stromal elements of the microcirculatory unit of fetal organs arise before the formation of their structural and functional units. In addition, active migration of mesenchymal cells during organogenesis provides spatial orientation of developing organs by marking their volume boundaries through restriction of homeotic Hox-types. The stromal framework also serves as the basis for the assembly of structural and functional units of parenchymatous organs, which often include morphogenetically and functionally completely different cells. Consequently, during embryogenesis, the functions of the mesenchyme are primary and are realized through the generation of regulatory signals that activate regional proliferation and differentiation of progenitor epithelial cells. Embryonic mesenchyme cells produce growth factors such as HGF-b, HGF-b, CSF, for which parenchymal progenitor cells have corresponding receptors. In differentiated mature tissue of an adult organism, the stromal network of cells also generates signals to maintain the viability and proliferation of progenitor cells of non-mesenchymal origin. However, the spectrum of stromal regulatory signals in postnatal ontogenesis is different (SCF, HGF, IL-6, IL-1, IL-8, IL-11, IL-12, IL-14, IL-15, GM-CSF, flt-3, LIF, etc.) and is aimed at ensuring physiological regeneration or repair of damaged tissue zones. Moreover, the spectral characteristics of stromal regulatory factors in each type of tissue and even within one organ are different. In particular, hematopoiesis and lymphopoiesis with the proliferation and differentiation of hematopoietic and immunocompetent cells occur only in certain organs, within the boundaries of which the stromal microenvironment operates, providing conditions for the maturation of hematopoietic and lymphoid cells. The ability of hematopoietic and lymphoid cells to repopulate a given organ, proliferate and mature in its microstructural niches depends on the regulatory factors of the microenvironment.
Among the components of the extracellular matrix that multipotent mesenchymal progenitor cells produce, fibronectin, laminin, collagen and proteoglycans, as well as CD44 (hyaluronan and osteopontin receptor), should be noted, which take a major part in organizing intercellular interactions and forming the extracellular matrix in the bone marrow and bone tissue. It has been proven that bone marrow multipotent mesenchymal progenitor cells create a stromal microenvironment that provides inductive and regulatory signals not only to MSCs, but also to hematopoietic progenitors and other non-mesenchymal stem cells of the bone marrow. It is known that the participation of MSCs in hematopoiesis is determined by their ability to differentiate into stromal cells that support hematopoiesis, and this instructive signal is received by MSCs directly from hematopoietic stem cells. This is why in culture the network of stromal progenitor cells serves as a feeder base for the development of all clones of hematopoietic cells.
In a mature organism, the intensity of hemo- and lymphopoiesis is in a state of dynamic equilibrium with the “expenditure” of mature blood cells and immune system cells on the periphery. Since stromal cells of the bone marrow and lymphoid organs are renewed extremely rarely, significant restructuring of stromal structures in them does not occur. The system can be taken out of dynamic equilibrium by mechanical damage to any of the organs of hemo- or lymphopoiesis, which leads to uniform sequential changes that concern not only and not so much the hematopoietic or lymphoid elements as the stromal structures of the damaged organ. In the process of reparative regeneration, the stromal base is formed first, which is then repopulated by hematopoietic or immunocompetent cells. This long-known fact makes post-traumatic regeneration a convenient model for studying the stromal microenvironment of hematopoietic organs. In particular, mechanical emptying of the medullary cavity of tubular bones is used to study reparative regeneration of bone marrow - curettage, which allows for quick and effective removal of hematopoietic tissue from the state of dynamic equilibrium. When studying the processes of reparative regeneration of the hematopoietic and stromal components of bone marrow after mechanical emptying of the medullary cavity of the tibia of guinea pigs, it was found that there is no direct correlation between the indices of regeneration of hematopoietic and stromal cells (the number of hematopoietic cells, the concentration and number of stromal progenitor cells). In addition, it turned out that an increase in the population of stromal progenitor cells occurs at an earlier time after curettage, and the stromal fibroblasts themselves become phosphatase-positive, which is typical of osteogenic tissue. It has also been established that curettage of 3-5 tubular bones leads to the growth of this cell population in the bone marrow of non-operated bones and even in the spleen, which in guinea pigs is an exclusively lymphopoietic organ.
The morphological picture of reparative processes in the bone marrow of curetted tibiae of guinea pigs generally corresponds to the data described in the literature obtained in experiments on animals of other species, and the dynamics of changes occurring after the removal of hematopoietic tissue is the same for all animal species and the difference concerns only time parameters. Morphologically, the phase order of hematopoiesis restoration in the emptied medullary cavity consists of successive processes of blood clot organization, formation of coarse fibrous bone tissue, its resorption, development of sinusoids and formation of reticular stroma, which is subsequently repopulated by hematopoietic elements. In this case, the number of progenitor hematopoietic cells in the process of bone marrow tissue regeneration increases in parallel with the increase in the content of hematopoietic stem cells.
Yu. Gerasimov and co-authors (2001) compared changes in the number of hematopoietic cells and the number of stromal progenitor cells in individual phases of the regeneration process. It turned out that quantitative changes in bone marrow cells in curetted bone correspond to the dynamics of the morphological characteristics of regeneration. The authors associate the decrease in the cellular content in the regenerate during the first three days with the death of hematopoietic cells due to the unfavorable effect of the microenvironment created by the proliferating reticular tissue in the preserved bone marrow in the epiphysis region, as well as with the formation of osteoid tissue foci in the latter and vascular damage during curettage. On the 7th-12th day, an increase in the level of nucleated cells coincides with the appearance of individual foci of myeloid hematopoiesis in the zones of proliferation of stromal elements. On the 20th day, significant areas of regenerated bone marrow and well-developed sinuses appear, which is accompanied by a significant increase in the total number of cells. However, the number of hematopoietic elements during this period is 68% of the control level. This is consistent with previously published data that the number of hematopoietic cells after curettage reaches the norm only on the 35th-40th day after surgery.
In the early post-traumatic period, the main source for the restoration of hematopoiesis are local cellular elements preserved during curettage. At later stages, the main source of regeneration of bone marrow hematopoietic tissue are stem cells repopulating free stromal zones. As for individual categories of stromal cells (endothelial, reticular and osteogenic), the sources that ensure their formation during the reorganization of the bone marrow cavity remain unclear. The results of the study by Yu. V. Gerasimov and co-authors (2001) indicate that in the bone marrow preserved after curettage, the concentration of cells forming colonies of fibroblasts is significantly higher than in normal bone marrow. The authors believe that curettage results in a more intensive selective washout of hematopoietic cells compared to colony-forming stromal cells, which participate in the formation of the stroma and are more strongly associated with its main substance than hematopoietic cells.
The dynamics of the change in the number of cells forming fibroblast colonies correlates with the intensity of osteogenesis processes, subsequent resorption of bone trabeculae and formation of reticular stroma, which is populated by hematopoietic cells. Most of the stromal progenitor cells in the specified terms of regeneration form coarse fibrous bone tissue and reticular stroma. In case of femoral fractures under conditions of prolonged osteosynthesis, on the 5th day in the regeneration zone the concentration and number of cells forming fibroblast colonies increases, and during the period of intensive bone formation their number increases 6 times. It is known that bone marrow cells forming fibroblast colonies have osteogenic properties. The number of stromal progenitor cells increases before the settlement of the curetted bone marrow territory by hematopoietic cells. This is in good agreement with the data that stromal cells provide the formation of a hematopoietic microenvironment. Apparently, the creation of a hematopoietic microenvironment corresponds to a certain level of stromal tissue regeneration, and the number of hematopoietic cells increases with the expansion of the stromal platform suitable for hematopoiesis.
Of greatest interest are the authors' data that immediately after curettage the number of stromal progenitor cells in the remote parts of the skeleton increases. Starting from the sixth hour and up to the twentieth day inclusive, a more than twofold increase in both the concentration and the number of cells forming fibroblast colonies is observed in the contralateral tibia. The mechanism of this phenomenon is probably associated with the fact that massive bone marrow injury leads to the formation of a large number of blood clots with the simultaneous destruction of a significant number of platelets and the release of platelet-derived growth factor (PDGF) into the blood, which is known to cause proliferation of cells forming fibroblast colonies located in the body outside the proliferative pool. In experiments on rabbits, local administration of MSCs promotes the restoration of cartilage tissue of the surgically damaged knee joint, which can be associated with the formation of chondrocytes originating from the injected MSCs. However, reparative regeneration of bone defects in laboratory rats is significantly enhanced by using mesenchymal stem cells enclosed in a ceramic framework. Therefore, it can be assumed that if not RBOC, then some other factor originating from damaged stromal cells exerts a distant stimulating effect on the proliferation of mesenchymal progenitor cells in intact bone marrow zones and stimulates their migration to the bone marrow defect area. In turn, this is contradicted by literature data from previous years indicating that stromal cells responsible for the microenvironment, unlike hematopoietic cells, are not capable of migration and originate from local sources.
Nevertheless, the results of the study by Yu. Gerasimov and co-authors (2001) indicate that mechanical trauma causes not only a sharp restructuring of the stromal tissue in the curetted bone, but also significant changes in the stroma in distant intact bones, i.e., there is a systemic response of the stromal tissue to local trauma. Moreover, when polytrauma is inflicted - multiple curettage - this reaction is enhanced and is observed not only in the operated bone and distant parts of the skeleton, but also in the lymphoid organs, in particular in the spleen. The mechanism of such a systemic response of the stromal tissue of the bone marrow and spleen to local trauma and polytrauma remains unknown. It is assumed that this process is associated with the action of a humoral factor secreted by the mesenchymal stroma of the medullary cavity of the bone marrow. The possibility of the production by stromal cells of the bone marrow and spleen of an organ-nonspecific humoral factor responsible for the proliferation of cells that form fibroblast colonies is evidenced by data on their colony-stimulating activity in monolayer cultures of bone marrow.
In this regard, it is worth noting that with systemic administration of multipotent mesenchymal progenitor cells, their derivatives repopulate not only bone marrow, but also other tissues, which is used, in particular, for gene therapy. It has been shown that with intravenous administration of large quantities of MSCs with a wild-type genome to mice with a mutation in the collagen I gene, donor cells replace up to 30% of cells in the bone and cartilage tissue of recipients, and transfected mouse mesenchymal stem cells secreting human IL-3 effectively support hematopoiesis for 9 months in the case of their simultaneous administration with human hematopoietic stem cells to immunodeficient mice.
[ 3 ], [ 4 ], [ 5 ], [ 6 ], [ 7 ], [ 8 ], [ 9 ], [ 10 ]
Genetic modification of mesenchymal stem cells
Among the successes of experimental genetic modification of MSCs, it is worth noting the transfection of the factor IX gene into human MSCs with subsequent transfer of transfectant cells to immunodeficient mice, which leads to the appearance of antihemophilic factor B in the blood for 8 weeks after transplantation. In this experiment, post-translational modification of factor IX by y-glutamyl carboxylase was carried out in the transfected cells. Transduction of MSCs with a retroviral vector encoding human factor IX was less successful - subsequent administration of these cells to a dog with hemophilia B provided a therapeutic level of factor IX, maintaining normal intensity of coagulation hemostasis, for only 12 days.
Transplantation of mesenchymal stem cells into the brain parenchyma of animals has shown that donor immature cells are transformed into both neuronal and glial populations. Engraftment of neuronal derivatives of healthy donor mesenchymal tissue theoretically makes it possible to correct genetic abnormalities of brain metabolism in patients with Gaucher disease and other disorders of lipid, ganglioside, or carbohydrate metabolism.
The experimental search for conditions for transdifferentiation of bone marrow stromal stem cells into neural and liver tissue progenitor cells is ongoing. The researchers' attention is focused on combinations of differentiation inducers and special conditioned media. In particular, to isolate the primary culture of stromal cells, bone marrow cells washed and resuspended in DMEM/F12 (1/1) culture medium with 10% fetal calf serum are seeded at a density of 200,000/cm2. After 24 hours, non-adherent cells are removed, and fibroblast-like cells attached to the plastic are cultured for one week. For differentiation of bone marrow stromal cells into neuroblasts, a conditioned medium obtained by three-day cultivation of the primary culture of mouse embryonic fibroblasts is used, as well as DMEM/F12 medium (1/1) with 2% fetal calf serum and the addition of 20 ng/ml NF or 10-6 M retinoic acid (neuroinducers that are used for neural differentiation of mouse and human embryonic stem cells). Differentiation of bone marrow stromal cells into hepatocyte precursor cells is induced in a conditioned medium created as a result of three-day cultivation of the primary culture of mouse embryonic liver cells in DMEM/F12 medium (1/1) with the addition of 10% fetal calf serum.
Here it should be noted once again that the colony-forming cells of the bone marrow stroma are heteromorphic and can be divided into two types. The first type includes fibroblast-like cells that form filopodia with large nuclei and one or two nucleoli. The second type is represented by small spindle-shaped cells. When culturing cells of both types in a conditioned medium obtained on a feeder layer of primary mouse embryonic fibroblasts, cells similar to neuroblasts appear in the culture on the 3rd to 4th day. At this stage, they most often have a spindle-shaped form with one or two long processes ending in filopodia. Less common are pyramidal or stellate cells with short dendrites. The dendrites of some neuroblasts have characteristic expansions (growth buds) and branches in their distal part, while others have distinct growth cones with filopodia, through which the dendrites grow. Similar morphological features (buds and growth cones with filopodia) inherent in neuroblasts differentiating into neurons have been described in detail in studies on neurogenesis. Based on this, some authors conclude that the cells they found in the culture are neuroblasts. In particular, E. Shchegelskaya and co-authors (2002) after culturing a primary culture of stromal cells for two weeks in a conditioned medium changed every 3rd to 4th day found that some of the cells proliferated while maintaining an undifferentiated state. Outwardly, such cells resembled fibroblasts and were detected in the culture along with differentiating neuroblasts. The majority of the cells (about 80%) were at different stages of differentiation into cells of the nervous tissue, primarily into neurons. The dendritic processes of these cells were in close contact with each other, so that the cells gradually formed sections of the nervous network on the substrate in the form of long multicellular strands. The dendritic processes of neuroblasts became significantly longer, some of them exceeded the length of the neuron body itself by 8-10 times. The proportion of pyramidal and stellate cells gradually increased. The dendrites of stellate cells branched. According to the authors, the later differentiation of pyramidal and stellate cells compared to spindle-shaped cells corresponds to the sequence of stages of normal neurogenesis in animals. As a result, the authors conclude that bone marrow stromal stem cells undergo induced neurogenesis, during which all three main types of neurons are formed from neuroblasts in vitro. Nerve cell precursors were also detected during cultivation of bone marrow stromal cells for 3-4 days in a medium with 2% fetal serum and 20 ng/ml LIF. But in this case, stem cells divided very slowly, neuroblast differentiation occurred only in 30% of cases and they did not form neuronal networks. Using retinoic acid as one of the inducers of nerve cell differentiation,The authors obtained up to 25-30% of nerve cells in the culture, with glial elements predominantly - astrocytes and oligodendrocytes. Neurons constituted only a third of all nerve cells, although they were represented by all three types: spindle-shaped, pyramidal and stellate cells. On the 6th day of culturing stromal cells in a medium with retinoic acid, nerve cells became more differentiated, and axons were found in individual pyramidal neurons, which in normal neuroontogenesis appear later than the formation of dendritic processes. According to the authors, despite the low yield of nerve cells, the retinoic acid induction method has its advantages: oligodendrocytes and astrocytes perform myelinating and nutritional functions during the growth of dendrites and axons and are necessary for the normal formation of nerve tissue. Therefore, for the repair of its damaged areas in vivo, it is better to use a suspension of neurons enriched with glial cells.
In the second series of experiments, the authors attempted to induce differentiation of bone marrow stromal cells into liver cells. After three days of culturing bone marrow stromal stem cells in a conditioned medium obtained by incubating mouse embryonic hepatocytes, large, spherical cells were found, often binuclear, with cytoplasmic inclusions of varying sizes. These cells were at different stages of differentiation and differed in size, number of nuclei, and inclusions in the cytoplasm. Glycogen was detected in most of these cells, on the basis of which the authors identified them as hepatocyte precursor cells. Since no cells similar to neuroblasts were found in the culture, it was concluded that the conditioned medium obtained as a result of culturing embryonic hepatocytes lacked differentiation factors of nerve cells and, conversely, contained factors inducing differentiation of bone marrow stromal cells into hepatocyte precursor cells. In conclusion, the authors suggest the presence of pluripotency in bone marrow stromal cells, since they differentiate in vitro into nerve or liver tissue cells depending on the specific conditioned media and inducers used.
Some studies have indeed shown correctly the differentiation of bone marrow stromal cells into cardiomyocytes, cartilage, bone and nerve tissue cells. There is evidence that among bone marrow cells there are populations of stem cells capable of differentiating into hepatocytes. In light of these data, the results of the above experiments on mice can still be considered further confirmation of the presence in the bone marrow of pluripotent mesenchymal stem cells capable of differentiating into cells of various tissues of an adult organism.
Mesenchymal stem cell transplantation
In clinical transplantology, human mesenchymal stem cells can be used to ensure the expansion of hematopoietic stem cells, as well as their early precommitted descendants. In particular, the introduction of autologous hematopoietic stem cells and MSCs to cancer patients after high-dose chemotherapy accelerates the restoration of the number of neutrophils and platelets in the peripheral blood. Allo- and autologous transplants of mesenchymal stem cells are used to treat multiple myeloma, aplastic anemia and spontaneous thrombocytopenia - diseases associated with a primary defect in the stroma of hematopoietic tissue. The efficiency of cell therapy in oncohematological pathology is in many cases higher with the simultaneous introduction of stromal and hematopoietic stem cells, which is manifested by a reduction in the postoperative period of hematopoiesis restoration, a decrease in the number of fatal outcomes due to non-selective destruction of regional and circulating cancer cells, in which the patient's own progenitor hematopoietic cells also die. The prospects of using MSCs and other multipotent mesenchymal progenitor cells in clinical practice are due to the relative ease of obtaining them from bone marrow aspirates, expansion in culture and transfection of therapeutic genes. At the same time, local implantation of multipotent mesenchymal progenitor cells can be used to compensate for local tissue defects, and in case of systemic dysfunctions of tissues of mesenchymal origin, their introduction into the general bloodstream is not excluded.
The authors of works, in which the prospects for the use of MSCs for local, systemic transplantation and gene therapy are analyzed from the point of view of stromal cell biology, are more cautious in their reasoning. Postnatal bone marrow is traditionally considered as an organ consisting of two main systems of clearly defined cell lines - the hematopoietic tissue itself and the supporting stroma associated with it. Therefore, bone marrow mesenchymal stem cells were initially regarded exclusively as a source of stromal basis for the production of regulatory factors of the hematopoietic microenvironment. Then the attention of researchers switched to studying the role of MSCs as a stem source of skeletal tissues. The latest data indicate an unexpected potential for the differentiation of bone marrow stromal cells with the formation of neural or muscle tissue. In other words, mesenchymal stem cells exhibit transgermal plasticity - the ability to differentiate into cell types phenotypically unrelated to the cells of the original tissue. At the same time, some aspects of the biology of bone marrow stromal cells remain unclear and unresolved both in general biological terms and in individual details, including the identification, nature, origin, development and function in vivo of bone marrow stromal cells, as well as the permissible differentiation potential ex vivo and the possibilities of therapeutic use in vivo. The data obtained on the potential of MSCs, as well as the results of studies of the regenerative potential of other stem cells, are in sharp contradiction with the dogmas established in biology.
When cultured at low density, bone marrow stromal stem cells form distinct colonies, each derived from a single progenitor cell. The percentage of stromal progenitor cells in nucleated bone marrow cells, determined by colony-forming ability, is highly dependent on both culture conditions and MSC species. For example, in rodents, the presence of irradiated bone marrow feeder cells and serum in the culture is absolutely necessary to obtain the maximum number of stromal progenitor cells, whereas in humans, the colony-forming efficiency of mesenchymal stem cells is independent of both the feeder and the culture medium. The number of known mitogenic factors that stimulate stromal progenitor cell proliferation is limited. These include PDGF, EGF, FGF, TGF-b, and IGF1. Under optimal culture conditions, polyclonal MSC lines can withstand more than 50 cell divisions in vitro, making it possible to obtain billions of bone marrow stromal cells from 1 ml of its aspirate.
However, the population of bone marrow stromal cells is heterogeneous, which is manifested by both variability in colony sizes, different rates of their formation, and a variety of cellular morphology, which covers the range from fibroblast-like spindle-shaped to large flat cells. During the development of such cultures, phenotypic heterogeneity is also noted after 20 days. Some colonies are characterized by high expression of alkaline phosphatase, others do not express it at all, and colonies of the third type are phosphatase-positive in the central region and phosphatase-negative at the periphery. Individual colonies form nodules of bone tissue (the onset of matrix mineralization is marked by staining with alizarin red or for calcium according to Van Koss). In other colonies, fat accumulation occurs, identified by G-staining with oil red. Less often, colonies of mesenchymal stem cells form cartilages stained with Alcian blue).
After ectopic transplantation into experimental animals, polyclonal MGK lines form ectopic bone with a reticular stroma associated with myelopoiesis and adipocytes, and, less commonly, with cartilage tissue. When monoclonal lines of bone marrow stromal cells are transplanted, chimerism is observed in some cases, in which the de novo bone consists of bone tissue cells, contains stroma and adipocytes of donor origin, while the cells of the hematopoietic lineage and the vascular system are derived from the recipient.
The results of these studies confirm the stem nature of the bone marrow stromal progenitor cell from which the clonal line was derived. They also indicate that not all cells clonogenic in culture are truly multipotent stem cells. Some researchers believe, and we share their opinion, that the most reliable information on the real differentiation potential of individual clones can only be obtained in vivo after transplantation, and not by determining the phenotype of their derivatives in vitro. The expression in culture of phenotypic markers of osteo-, chondro-, or adipogenesis (determined by mRNA or by histochemical techniques) and even the production of mineralized matrix do not reflect the degree of pluripotency of an individual clone in vivo. Therefore, the identification of stem cells in a group of stromal cells is possible only a posteriori, under the appropriate conditions of a biological transplantation assay. In particular, chondrogenesis is very rarely observed in open transplantation systems, whereas cartilage formation is far from uncommon in closed systems such as diffusion chambers or in vitro micromass cultures of stromal cells, where local low oxygen tension is achieved, which promotes the formation of cartilage tissue. Therefore, even the transplantation technique, as well as non-specific in vitro culture conditions, significantly affect the range of MSC differentiation.
Experimental transplantation under specified experimental conditions is the gold standard for determining the differentiation potential of bone marrow stromal cells and a key element in their identification. Historically, studies on bone marrow stromal cell transplantation are associated with the general problem of bone marrow transplantation. It has been established that the hematopoietic microenvironment is created by transplanting bone marrow stromal cell lines and provides ectopic development of hematopoietic tissue in the transplantation zone. The origin of the microenvironment from the donor, and the hematopoietic tissue from the host allows us to consider ectopic bone as a true “inverted” bone marrow transplantation. Local transplantation of bone marrow stromal cells promotes effective correction of bone defects, more pronounced than with spontaneous reparative regeneration. Several preclinical studies on experimental models have convincingly demonstrated the possibility of using bone marrow stromal cell transplants in orthopedics, although the most careful work and analysis are needed to optimize these methods, even in the simplest cases. In particular, the optimal conditions for the expansion of osteogenic stromal cells ex vivo have not yet been established, the structure and composition of the ideal carrier, as well as the number of cells necessary for volumetric bone regeneration, remain undeveloped.
In addition to the use of ex vivo expanded bone marrow stromal cells for the regeneration of tissues of mesenchymal origin, the unorthodox plasticity of MSCs opens up potential applications for the regeneration of neural cells or the delivery of gene products to the CNS. In principle, this simplifies cell therapy for nervous system damage, since there is no need to obtain autologous human neural stem cells. Potential applications of bone marrow cells for the generation of cardiomyocytes and myogenic progenitor cells of both true stromal and extrastromal origin have been reported.
Experiments are being conducted on systemic transplantation of bone marrow stromal cells for the treatment of common skeletal diseases. There is no doubt that bone marrow stromal cells are the population responsible for genetic disorders in skeletal diseases, which is well illustrated by the vector transfer of genetic information using these cells, which leads to the formation of pathological bone tissue in experimental animals. However, the ability of stromal cells to implant, engraft, proliferate and differentiate in skeletal bones after introduction into the general bloodstream has not yet been proven.
This is partly because in standard bone marrow transplantation the stroma is not transplanted together with the hematopoietic tissue, so strict criteria for assessing the successful engraftment of systemically administered stromal cells have yet to be developed. It should be remembered that the presence of marker genes in tissue extracts or the isolation of cells of donor origin in culture does not indicate engraftment of the cells, but only their survival. Even intra-arterial injection of bone marrow stromal cells into a mouse limb can lead to virtually zero engraftment, despite the fact that cells of donor origin are found in large numbers within the bone marrow microvasculature. Unfortunately, such cells are usually described as “engrafted” simply on the basis of the detection of marker genes for donor cells in ex vivo culture. In addition, convincing evidence of long-term integration of differentiated and functionally active cells of donor origin in the tissues under study must be provided. In many published papers reporting on the engraftment of bone marrow stromal cells in the skeleton, the absence of clear data of this kind is striking. However, it should be noted that some correct animal experiments have indeed established a limited but real engraftment of stromal progenitor cells after their systemic administration.
These data are consistent with the results of studies on the possibility of delivering bone marrow myogenic progenitor cells to muscle via the vascular system. However, it should not be forgotten that both skeletal and muscle tissue are formed during development and growth based on extravascular cell movements that use migration processes that do not involve blood circulation. If an independent circulatory pathway for delivering progenitor cells to solid phase tissues does exist, is it possible to assume the existence of physiologically circulating mesenchymal progenitor cells? What is the origin of these cells in both the developing and postnatal organism, and how do they penetrate the vascular wall? The solution of these questions seems absolutely necessary and requires the most careful preclinical analysis. Even after answers to these questions are found, problematic kinetic aspects associated with skeletal growth and connective tissue remodeling will remain unresolved. At the same time, the treatment of osteogenesis disorders by replacing the entire population of mutated skeletal progenitor cells with healthy stromal elements appears to be a real clinical prospect. In this case, local fracture zones or deformations due to pathological osteogenesis, as well as destructive changes in bone tissue, can be corrected using stromal stem cells cultured in vitro. Therefore, it is advisable to focus future research on the problems of transformation or genetic correction of autologous mutated osteogenic progenitor cells ex vivo.
Genetic engineering of cells, short-term or permanent, has become the basis of cellular and molecular biology, the source of many scientific discoveries concerning the role of individual proteins in cellular metabolism in vitro and in vivo. The use of molecular technologies for the correction of hereditary pathology and human diseases is very promising for practical medicine, since the properties of bone marrow stromal stem cells make it possible to develop unique transplantation schemes for the correction of genetic diseases of the skeleton. At the same time, mesenchymal precursor cells can be easily obtained from the future recipient, they are amenable to genetic manipulation and are capable of multiplying in large quantities in a short period of time. The use of mesenchymal stem cells allows one to avoid the limitations and risks associated with the delivery of genetic information material directly to the patient through intravascular vector constructs. A similar strategy is applicable to embryonic stem cells, but autologous postnatal bone marrow stromal cells are a more preferable material, since their introduction excludes possible immunological post-transplant complications. To achieve a short-term effect, for example, to accelerate bone regeneration, the most optimal method is genetic modification of mesenchymal stem cells using electroporation, chemical fusion, lipofection, plasmids and adenoviral constructs. In particular, viral transfection into bone marrow stromal cells BMP-2 has proven effective in accelerating bone regeneration in experimental polytrauma. The creation of adenoviral vector constructs is preferable due to the absence of toxicity. However, genetic modification of bone marrow stromal cells in this case is characterized by extremely low stability. In addition, normal transformed bone marrow stromal cells require the use of vector carriers of genetic information that are 10 times more infectious than other cell types, which significantly increases the percentage of death of transfected cells.
Treatment of recessive diseases caused by low or zero biological activity of certain genes requires long-term or permanent modification of mesenchymal stem cells, which requires the use of adeno-associated viruses, retroviruses, lentiviruses or adeno-retroviral chimeras. The transport regions of these viruses are capable of transferring large DNA transfects (up to 8 kb). The scientific literature has already reported on the exogenous biological activity of bone marrow stromal cells transfected with retroviral constructs encoding the synthesis of regulatory and marker molecules - IL-3, CD2, factor VIII, as well as enzymes involved in the synthesis of L-DOPA. However, even in these studies, the authors point out a number of limitations that need to be overcome before the practical application of this technology. The first problem is to optimize the process of MSC modification ex vivo. It is known that long-term (3-4 weeks) proliferation of bone marrow stromal cells in vitro reduces their transfection. At the same time, to achieve a high level of genetic modification of MSCs, it is necessary to carry out several transfection cycles. The second problem is associated with the duration of therapeutic gene expression, which does not exceed four months yet. A natural decrease in effective gene expression is due to promoter inactivation and death of modified cells. With the general prospects of transferring genetic information using mesenchymal stem cells, the results of preliminary studies indicate the need for further optimization of ex vivo transfection methods, the choice of an adequate promoter regulating biological activity in the desired direction, and an increase in the ability of modified bone marrow stromal cells to self-maintenance in vivo after transplantation. It should be noted that the use of retroviral constructs for modifying bone marrow stromal cells in the desired direction does not always require their mandatory engraftment. Transfected mesenchymal stem cells can perform a corrective function against the background of stable residency and without mandatory active physical incorporation and functioning in connective tissue. In this case, they should be considered as a biological mini-pump producing in vivo a factor, the deficiency of which determines the manifestation of genetic pathology.
The use of transformed bone marrow stromal cells for the treatment of dominant genetic pathology, which is characterized by the expression of a gene with pathological or abnormal biological activity, is much more problematic, since in this case it is necessary to block the transfer or implementation of distorted genetic information. One of the methods of genetic engineering is homologous recombination of embryonic stem cells in order to create transgenic animals. However, the extremely low degree of homologous recombination in combination with the problems of identification, separation and expansion of such recombinants is unlikely to contribute to the widespread use of this method in the near future, even if new technological methods are developed. The second approach in gene therapy of dominant pathology is based on the automatic correction of damaged DNA, since genetic mutations can be corrected by introducing exogenous DNA with the desired sequence (short DNA oligonucleotides or chimeric RNA/DNA oligonucleotides), which binds to homologues in the damaged genome. The third option involves blocking the transmission of pathological information, which is achieved through the use of specifically designed oligonucleotides that bind to a specific gene to form a ternary helical structure that eliminates the possibility of transcription.
Although correction of a genetic disease at the genome level remains the most optimal and preferred therapeutic method, mRNA is also a promising vector (possibly even more accessible) for blocking a dominant negative gene. Protein molecules with antisense oligonucleotide or complete sequences that block mRNA binding to the cellular biosynthetic apparatus have long been used to inhibit translation and/or increase mRNA degradation. In addition, double-stranded RNA induces rapid mRNA degradation, the mechanism of which remains unclear. However, it is unlikely that the mere elimination of mRNAs transcribed from a mutant allele with short or single mutations will promote the expression of mRNA of the normal allele. An alternative is the use of hammerhead and hairpin ribosyntheses, which have the ability to bind to highly specific regions of mRNA with subsequent induction of their cleavage and inactivation during translation. The possibility of using this method in the therapy of pathological osteogenesis is currently being studied. Regardless of what exactly is the target - genomic or cytoplasmic elements, the success of new gene therapy technologies will be determined by the efficiency of the inclusion of reagents in bone marrow stromal cells ex vivo, the optimal choice of a specific vector and the stable ability of mesenchymal stem cells to express the necessary factors in vivo.
Thus, the discovery of mesenchymal stem cells with their unexpected properties creates a new conceptual scheme for the development of cell lines. However, further interdisciplinary research is needed to understand the biological role of stromal stem cells, their nature, their ability to transdifferentiate or dedifferentiate, their physiological significance during embryonic development, postnatal growth, maturation and aging, as well as in human diseases.